Monday Article #78: Molecular Adaptations to Exercise
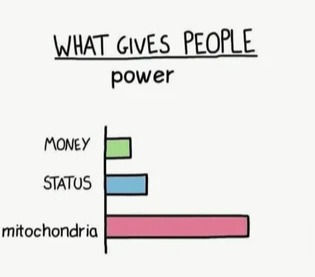
Mind’s racing, heart’s pumping, you’re panting: “Huff, puff, blah, oof, ew”. The breath of exhaustion radiates throughout the surroundings as you come to a stop after a 3-kilometer run. You think to yourself: I’m never going to do this again. The next month, you did it again, but this time, you felt like you could run another lap. What happened?! Did your legs get longer or did you grow another heart? Magic, or is it?
Beyond your visible sweat, and fatiguing exertion, lies a hidden world of cellular transformation, where the microscopic choreography of genes, proteins, and many more molecules synchronize to elicit wonderful adaptations to exercise. Join me, as we unfold the miraculous ballet of molecular adaptations to exercise.
Of course, we won’t have enough space to discuss every single molecule related to adaptations to exercise, so here we discuss the more crucial ones.
The role of protein kinases
By far, one of the most understood molecular mechanisms of adaptation involves adenosine monophosphate kinase (AMPK), which is activated when cellular energy balance is disrupted such as during exercise (Chen et al., 2003). During recovery, our body cells need glucose to rebuild itself and incur molecular adaptations. AMPK seems to play a role in promoting glucose uptake during recovery from exercise by phosphorylating Tbc1d1 protein. This protein acts to delay the glucose transporter GLUT4’s removal from the plasma membrane (Kjøbsted et al., 2019). As a result of higher GLUT4, muscle cells are able to uptake more glucose and produce more energy (Stöckli, Fazakerley and James, 2011). However, some studies using genetic models suggest that in mice that lack AMPK, upregulation of GLUT4 expression still occurs. This, however, was countered by the fact that increasing AMPK levels increased GLUT4 expression in response to chronic exercise (GONG et al., 2011). This could be explained by the fact that, in biology, there are often multiple, complex, and redundant pathways that regulate activity in the body. In mice genetically modified to not have AMPK, exercise activates protein kinase D, which has similar downstream activities of AMPK, but is not activated in wild-type mice (McGee et al., 2014). Hence, overexpression of AMPK could increase adaptations, while underexpression does not affect adaptations due to compensatory mechanisms.
Conversely, AMPK seems to play an indispensable role in mitochondrial biogenesis, evident by AMPK-deficient mice having reduced mitochondrial biogenesis (the process of creating new mitochondria), content, and function (Lantier et al., 2014), as well as less increase in mitochondrial function in response to exercise (Brandauer et al., 2015). AMPK can phosphorylate PGC-1α, leading to mitochondrial biogenesis (Akimoto et al., 2005). Mitophagy (essentially recycling of the mitochondria) is also promoted by AMPK through its activation of the protein kinase Ulk1 (Laker et al., 2017). Together, these mechanisms seem to promote mitochondrial function as well as increase mitochondrial content.
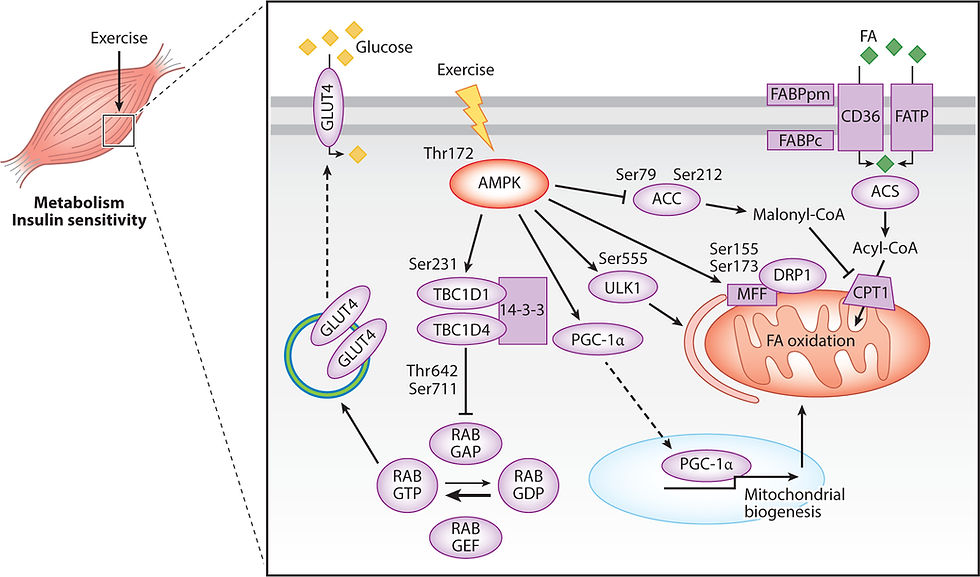
Figure 1: Molecular mechanisms of adaptations involving AMPK. Image taken from AMPK and the Adaptation to Exercise (Spaulding and Yan, 2022).
In a similar fashion, the calcium-calmodulin-dependent protein kinase II (CaMKII) is also activated by elevated cytoplasmic calcium levels, which is present during muscle contractions (Rose and Hargreaves, 2003). CaMKII increases DNA transcription by exporting histone deacetylases (HDAC) out from the nucleus. By removing HDACs, MEF2, a transcriptional activator binds onto the GLUT4 gene, which increases the expression of the GLUT4 gene (Grozinger and Schreiber, 2000). MEF2 also seems to increase PGC-1α expression (Jung and Kim, 2014).

Figure 2: CaMKII-mediated nuclear export of HDACs that allows MEF2 target genes to be transcribed. Image taken from Histone Deacetylase 5 Acquires Calcium/Calmodulin-Dependent Kinase II Responsiveness by Oligomerization with Histone Deacetylase 4 (Backs et al., 2008).
The role of chemokines
Contracting skeletal muscle can release metabolites known as myokines, which have a key role in intermediary metabolism during exercise. For example, the most common exercise-associated molecule is perhaps lactate, which can act as a signaling molecule to activate downstream processes such as hypoxia-inducible factor (HIF-1α) and vascular endothelial growth factor (VEGF) (Hunt et al., 2007). HIF-1α has been shown to upregulate the mRNA and protein content of MCT4 (Ullah, Davies and Halestrap, 2006). Higher levels of MCT4 allow fast-twitch muscle cells to export lactate and hydrogen ions (which reduces muscle pH) more effectively, allowing for higher-intensity exercise as the muscle won't get acidic as fast. HIF-1α also increases the expression of glucose transporters, specifically GLUT4 in muscle cells, which facilitates glucose entry into the cell for energy production (Sakagami et al., 2014).
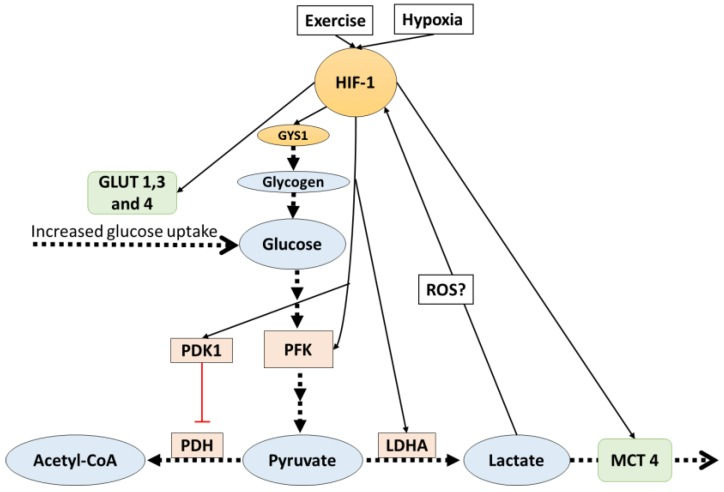
Figure 3: Potential HIF-1 mechanisms. Image taken from Lactate as a Signaling Molecule That Regulates Exercise-Induced Adaptations (Nalbandian and Takeda, 2016)
VEGF on the other hand is responsible for inducing angiogenesis (capillaries proliferation), which increases the surface area for glucose exchange. Increased oxygen and glucose delivery can then delay the onset of muscle fatigue during exercise (Joyner and Coyle, 2008).
Besides, lactate could also directly affect anabolism by promoting differentiation in muscle satellite cells, and activating the mammalian target of rapamycin (mTOR) (Oishi et al., 2015).
Aside from skeletal muscle-derived myokines, short-chain fatty acids produced by gut microbiota could also mediate some benefits of exercise in various organs (Scheiman et al., 2019).
The role of peroxisome proliferator-activated receptor gamma coactivator (PGC-1α)
Many adaptations to exercise are mediated by PGC-1α, which is widely accepted as the master controller of mitochondrial biogenesis. PGC-1α can be activated by a multitude of mechanisms as described earlier and allows for endurance exercise-induced expression of mitochondrial enzymes such as cytochrome c (Geng et al., 2010), as well as acts as a coactivator for various transcription factors involved in mitochondrial biogenesis (Lira et al., 2010). High expression of PGC-1α, usually associated with endurance training also enhances levels of mRNA for the slow oxidative-associated myosin heavy chain isoform, while reducing mRNA levels of the fast glycolytic-associated isoforms (Mortensen et al., 2006). This essentially leads to the conversion of fast-twitch muscle fibers into slow-twitch, particularly beneficial for endurance athletes.
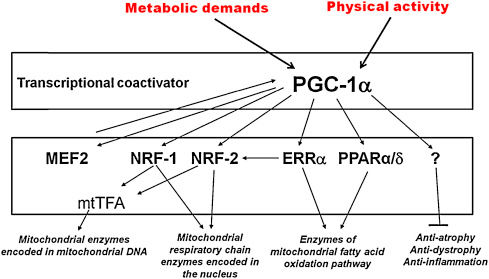
Figure 4: PGC-1α and its activated downstream transcription factors. Image taken from Exercise-induced PGC-1α transcriptional factors in skeletal muscle (Jung and Kim, 2014).
PGC-1α also upregulates the enzyme lactate dehydrogenase B which converts lactate into pyruvate (Summermatter et al., 2013). This step is particularly important for increasing the capacity for slow-twitch muscle fibers to oxidize fuel. PGC-1α seems to also increase the expression of MCT1 which is responsible for transporting lactate from the interstitial fluid into slow-twitch muscle fibers to be used as energy (Benton et al., 2008). Paradoxically, lactate also seems to activate PGC-1α as chronic suppression of lactate production reduces the PGC-1α-derived adaptations to high-intensity interval training (Hoshino et al., 2015). PGC-1α sorts of acts as the antagonist to HIF-1; PGC-1α mainly increases oxidative metabolism whereas HIF-1 increases glycolytic metabolism. Together, they can help increase the overall performance of an athlete by allowing more substrate utilization.

Figure 5: PGC-1α as a regulator and effector of lactate signaling. Image taken from Lactate as a Signaling Molecule That Regulates Exercise-Induced Adaptations (Nalbandian and Takeda, 2016)
The role of mammalian target of rapamycin (mTOR)
mTOR is the master regulator of protein synthesis and is heavily implicated in adaptations to strength training. Discussing all the pathways of mTOR would be more than enough for its own article, but the main takeaway is that mTOR can detect mechanical tension experienced by the muscle fibers and transmit it into a signal to increase protein synthesis, which is required for cell growth and regeneration (Wackerhage et al., 2019). mTOR, specifically mTOR complex I, increases protein synthesis by activating S6 kinase 1 and inhibiting 4E-binding protein 1 (a translation repressor protein) (Yoon, 2017). Activated S6 kinase 1 phosphorylates several residues of the ribosomal protein, increasing protein synthesis (Hideki Kawasome et al., 1998). All in all, the increased protein synthesis can lead to cellular growth and regeneration.
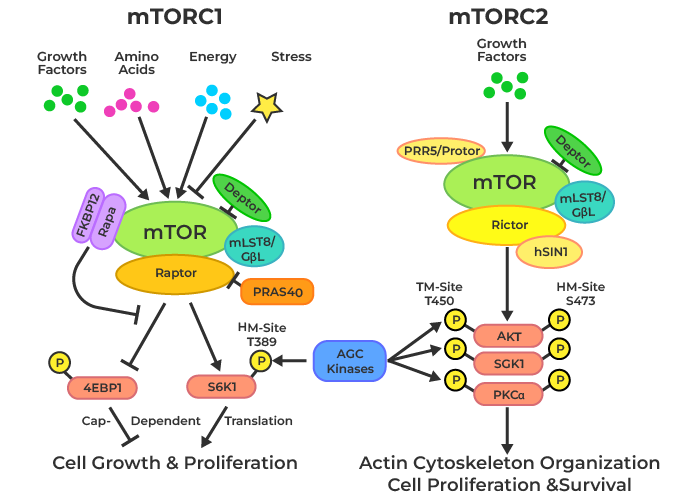
Figure 6: mTOR mechanisms. Image taken from Geeks For Geeks.
What does this all mean?
As mentioned earlier, biology possesses many wonderful redundant mechanisms of regulation, which means perhaps some of these molecular mechanisms are in fact sufficient but not necessary to cause exercise adaptations. In conclusion, all these molecular wonders lead to the various adaptations of exercise. This includes higher cardiorespiratory fitness, more efficient substrate utilization, higher muscle mass, better neuronal coordination, and many more that allow us to improve physically.
Article prepared by: Jared Ong Kang Jie, R&D Director of MBIOS 2023/2024
If you are interested in becoming a part of our MBIOS family and receiving our monthly newsletter, do sign up in the link below.
References
Akimoto, T., Pohnert, S.C., Li, P., Zhang, M., Gumbs, C., Rosenberg, P.B., Williams, R.S. and Yan, Z. (2005). Exercise Stimulates Pgc-1α Transcription in Skeletal Muscle through Activation of the p38 MAPK Pathway. Journal of Biological Chemistry, 280(20), pp.19587–19593. doi:https://doi.org/10.1074/jbc.m408862200.
Backs, J., Backs, T., Bezprozvannaya, S., McKinsey, T.A. and Olson, E.N. (2008). Histone Deacetylase 5 Acquires Calcium/Calmodulin-Dependent Kinase II Responsiveness by Oligomerization with Histone Deacetylase 4. Molecular and Cellular Biology, 28(10), pp.3437–3445. doi:https://doi.org/10.1128/mcb.01611-07.
Benton, C.R., Yoshida, Y., Lally, J., Han, X.-X., Hatta, H. and Bonen, A. (2008). PGC-1alpha increases skeletal muscle lactate uptake by increasing the expression of MCT1 but not MCT2 or MCT4. Physiological Genomics, [online] 35(1), pp.45–54. doi:https://doi.org/10.1152/physiolgenomics.90217.2008.
Brandauer, J., Andersen, M., Holti Kellezi, Risis, S., Frøsig, C., Vienberg, S.G. and Treebak, J.T. (2015). AMP-activated protein kinase controls exercise training- and AICAR-induced increases in SIRT3 and MnSOD. Frontiers in Physiology, 6. doi:https://doi.org/10.3389/fphys.2015.00085.
Chen, Z.-P. ., Stephens, T.J., Murthy, S., Canny, B.J., Hargreaves, M., Witters, L.A., Kemp, B.E. and McConell, G.K. (2003). Effect of Exercise Intensity on Skeletal Muscle AMPK Signaling in Humans. Diabetes, 52(9), pp.2205–2212. doi:https://doi.org/10.2337/diabetes.52.9.2205.
Geng, T., Li, P., Okutsu, M., Yin, X., Kwek, J., Zhang, M. and Yan, Z. (2010). PGC-1α plays a functional role in exercise-induced mitochondrial biogenesis and angiogenesis but not fiber-type transformation in mouse skeletal muscle. American Journal of Physiology-Cell Physiology, 298(3), pp.C572–C579. doi:https://doi.org/10.1152/ajpcell.00481.2009.
GONG, H., XIE, J., ZHANG, N., YAO, L. and ZHANG, Y. (2011). MEF2A Binding to the Glut4 Promoter Occurs via an AMPKα2-Dependent Mechanism. Medicine & Science in Sports & Exercise, 43(8), pp.1441–1450. doi:https://doi.org/10.1249/mss.0b013e31820f6093.
Grozinger, C.M. and Schreiber, S.L. (2000). Regulation of histone deacetylase 4 and 5 and transcriptional activity by 14-3- 3-dependent cellular localization. Proceedings of the National Academy of Sciences, 97(14), pp.7835–7840. doi:https://doi.org/10.1073/pnas.140199597.
Hideki Kawasome, Papst, P.J., Webb, S., Keller, G., Johnson, G.L., Gelfand, E.W. and Terada, N. (1998). Targeted disruption of p70s6kdefines its role in protein synthesis and rapamycin sensitivity. Proceedings of the National Academy of Sciences of the United States of America, [online] 95(9), pp.5033–5038. doi:https://doi.org/10.1073/pnas.95.9.5033.
Hoffman, Nolan J., Parker, Benjamin L., Chaudhuri, R., Fisher-Wellman, Kelsey H., Kleinert, M., Humphrey, Sean J., Yang, P., Holliday, M., Trefely, S., Fazakerley, Daniel J., Stöckli, J., Burchfield, James G., Jensen, Thomas E., Jothi, R., Kiens, B., Wojtaszewski, Jørgen F.P., Richter, Erik A. and James, David E. (2015). Global Phosphoproteomic Analysis of Human Skeletal Muscle Reveals a Network of Exercise-Regulated Kinases and AMPK Substrates. Cell Metabolism, 22(5), pp.922–935. doi:https://doi.org/10.1016/j.cmet.2015.09.001.
Hoshino, D., Tamura, Y., Masuda, H., Matsunaga, Y. and Hatta, H. (2015). Effects of decreased lactate accumulation after dichloroacetate administration on exercise training-induced mitochondrial adaptations in mouse skeletal muscle. Physiological Reports, 3(9), p.e12555. doi:https://doi.org/10.14814/phy2.12555.
Hunt, T.K., Aslam, R.S., Beckert, S., Wagner, S., Ghani, Q.P., Hussain, M.Z., Roy, S. and Sen, C.K. (2007). Aerobically Derived Lactate Stimulates Revascularization and Tissue Repair via Redox Mechanisms. Antioxidants & Redox Signaling, 9(8), pp.1115–1124. doi:https://doi.org/10.1089/ars.2007.1674.
Joyner, M.J. and Coyle, E.F. (2008). Endurance exercise performance: the physiology of champions. The Journal of Physiology, 586(1), pp.35–44.
Jung, S. and Kim, K. (2014). Exercise-induced PGC-1α transcriptional factors in skeletal muscle. Integrative Medicine Research, 3(4), pp.155–160. doi:https://doi.org/10.1016/j.imr.2014.09.004.
Kjøbsted, R., Roll, J.L.W., Jørgensen, N.O., Birk, J.B., Foretz, M., Viollet, B., Chadt, A., Al-Hasani, H. and Wojtaszewski, J.F.P. (2019). AMPK and TBC1D1 Regulate Muscle Glucose Uptake After, but Not During, Exercise and Contraction. Diabetes, 68(7), pp.1427–1440. doi:https://doi.org/10.2337/db19-0050.
Laker, R.C., Drake, J.C., Wilson, R.J., Lira, V.A., Lewellen, B.M., Ryall, K.A., Fisher, C.C., Zhang, M., Saucerman, J.J., Goodyear, L.J., Kundu, M. and Yan, Z. (2017). Ampk phosphorylation of Ulk1 is required for targeting of mitochondria to lysosomes in exercise-induced mitophagy. Nature Communications, [online] 8(1), p.548. doi:https://doi.org/10.1038/s41467-017-00520-9.
Lantier, L., Joachim Fentz, Mounier, R., Leclerc, J., Treebak, J.T., Pehmøller, C., Sanz, N., Sakakibara, I., Emmanuelle Saint-Amand, Stéphanie Rimbaud, Maire, P., Marette, A., Renée Ventura-Clapier, Ferry, A., Richter, E.A., Foretz, M. and Benoit Viollet (2014). AMPK controls exercise endurance, mitochondrial oxidative capacity, and skeletal muscle integrity. Federation of American Societies for Experimental Biology, 28(7), pp.3211–3224. doi:https://doi.org/10.1096/fj.14-250449.
Lira, V.A., Benton, C.R., Yan, Z. and Bonen, A. (2010). PGC-1α regulation by exercise training and its influences on muscle function and insulin sensitivity. American Journal of Physiology-Endocrinology and Metabolism, 299(2), pp.E145–E161. doi:https://doi.org/10.1152/ajpendo.00755.2009.
McGee, S.L., Swinton, C., Morrison, S., Gaur, V., Campbell, D.E., Sebastian Beck Jørgensen, Kemp, B.E., Baar, K., Steinberg, G.R. and Hargreaves, M. (2014). Compensatory regulation of HDAC5 in muscle maintains metabolic adaptive responses and metabolism in response to energetic stress. The FASEB Journal, 28(8), pp.3384–3395. doi:https://doi.org/10.1096/fj.14-249359.
Mortensen, O.H., Frandsen, L., Schjerling, P., Nishimura, E. and Grunnet, N. (2006). PGC-1alpha and PGC-1beta have both similar and distinct effects on myofiber switching toward an oxidative phenotype. American Journal of Physiology. Endocrinology and Metabolism, [online] 291(4), pp.E807-816. doi:https://doi.org/10.1152/ajpendo.00591.2005.
Nalbandian, M. and Takeda, M. (2016). Lactate as a Signaling Molecule That Regulates Exercise-Induced Adaptations. Biology, 5(4), p.38. doi:https://doi.org/10.3390/biology5040038.
Oishi, Y., Tsukamoto, H., Yokokawa, T., Hirotsu, K., Shimazu, M., Uchida, K., Tomi, H., Higashida, K., Iwanaka, N. and Hashimoto, T. (2015). Mixed lactate and caffeine compound increases satellite cell activity and anabolic signals for muscle hypertrophy. Journal of Applied Physiology, 118(6), pp.742–749. doi:https://doi.org/10.1152/japplphysiol.00054.2014.
Rose, A.J. and Hargreaves, M. (2003). Exercise Increases Ca2+-Calmodulin-Dependent Protein Kinase II Activity in Human Skeletal Muscle. The Journal of Physiology, 553(1), pp.303–309. doi:https://doi.org/10.1113/jphysiol.2003.054171.
Sakagami, H., Makino, Y., Mizumoto, K., Isoe, T., Takeda, Y., Watanabe, J., Fujita, Y., Takiyama, Y., Abiko, A. and Haneda, M. (2014). Loss of HIF-1α impairs GLUT4 translocation and glucose uptake by the skeletal muscle cells. American Journal of Physiology-Endocrinology and Metabolism, 306(9), pp.E1065–E1076. doi:https://doi.org/10.1152/ajpendo.00597.2012.
Scheiman, J., Luber, J.M., Chavkin, T.A., MacDonald, T., Tung, A., Pham, L.-D., Wibowo, M.C., Wurth, R.C., Punthambaker, S., Tierney, B.T., Yang, Z., Hattab, M.W., Avila-Pacheco, J., Clish, C.B., Lessard, S., Church, G.M. and Kostic, A.D. (2019). Meta-omics analysis of elite athletes identifies a performance-enhancing microbe that functions via lactate metabolism. Nature Medicine, [online] 25(7), pp.1104–1109. doi:https://doi.org/10.1038/s41591-019-0485-4.
Shibuya, M. (2011). Vascular Endothelial Growth Factor (VEGF) and Its Receptor (VEGFR) Signaling in Angiogenesis: A Crucial Target for Anti- and Pro-Angiogenic Therapies. Genes & Cancer, [online] 2(12), pp.1097–1105. doi:https://doi.org/10.1177/1947601911423031.
Spaulding, H.R. and Yan, Z. (2022). AMPK and the Adaptation to Exercise. Annual Review of Physiology, 84(1), pp.209–227. doi:https://doi.org/10.1146/annurev-physiol-060721-095517.
Stöckli, J., Fazakerley, D.J. and James, D.E. (2011). GLUT4 exocytosis. Journal of Cell Science, [online] 124(24), pp.4147–4159. doi:https://doi.org/10.1242/jcs.097063.
Summermatter, S., Santos, G., Pérez-Schindler, J. and Handschin, C. (2013). Skeletal muscle PGC-1α controls whole-body lactate homeostasis through estrogen-related receptor α-dependent activation of LDH B and repression of LDH A. Proceedings of the National Academy of Sciences of the United States of America, [online] 110(21), pp.8738–8743. doi:https://doi.org/10.1073/pnas.1212976110.
Ullah, M.S., Davies, A.J. and Halestrap, A.P. (2006). The Plasma Membrane Lactate Transporter MCT4, but Not MCT1, Is Up-regulated by Hypoxia through a HIF-1α-dependent Mechanism. Journal of Biological Chemistry, [online] 281(14), pp.9030–9037. doi:https://doi.org/10.1074/jbc.m511397200.
Wackerhage, H., Schoenfeld, B.J., Hamilton, D.L., Lehti, M. and Hulmi, J.J. (2019). Stimuli and sensors that initiate skeletal muscle hypertrophy following resistance exercise. Journal of Applied Physiology, 126(1), pp.30–43. doi:https://doi.org/10.1152/japplphysiol.00685.2018.
Yoon, M.-S. (2017). mTOR as a Key Regulator in Maintaining Skeletal Muscle Mass. Frontiers in Physiology, 8. doi:https://doi.org/10.3389/fphys.2017.00788.
Comments